Adult cardiomyocyte regeneration: if I could ever travel back time
Myocardial infarction (MI), also known as heart attack, affects more than three million people per year in United States and remains to be the leading cause of mortality world-wide due to lack of effective treatment (1). During acute MI, a large number of cardiomyocytes are lost by necrosis due to insufficient blood supply. Due to a lack of regenerative capacity of adult cardiomyocytes (ACM), these infarct areas are subsequently repopulated by fibrotic tissue, forming scar within the myocardium that contributes to eventual heart failure. Therefore, a major goal of the current research on acute MI is to re-supply cardiomyocytes population by inducing regenerative capacity in the adult heart.
Although adult mammalian heart is used to be considered as a post mitotic and terminally differentiated organ, recent exciting discoveries are challenging this traditional view. One such possibility is that, during the acute phase of cardiomyocyte loss, resident cardiac stem cells can be activated, and differentiate to give rise to new functional cardiomyocytes. Another possibility is the existing ACM can re-enter the cell cycle and proliferate at a very low speed to generate progenies. In fact, most of the studies testing this hypothesis have been focusing on boosting the proliferation efficiency via genetic manipulation, including Hippo pathway (2) and PPAR pathway (3). One potential caveat is, these studies failed to take into consideration the impact of cellular environment on cardiomyocytes differentiation and maturation, therefore, it is unclear what is the percentage of the newly generated daughter cells become mature cardiomyocytes with full contractile function. This limitation has been addressed by Wang et al. in their recent study (4), where they showed that ACM can go through a dedifferentiation, proliferation and redifferentiation cycle (ACM-DPR) post injury, raising new possibilities for regenerative therapy for injured myocardium in post MI hearts.
Prior to Wang et al., the proliferation of pre-existing ACM in human heart has indeed been documented by measuring mitochondria DNA content and cell cycle marker gene expression (5). However, the functional contribution and the therapeutic potential of these proliferating ACM to cardiac repair remains unclear as the turnover rate of human heart cardiomyocytes is very slow, ranging from 0.3–1% per year (6,7). That is, only a very small percentage of ACM has proliferation capacity to regenerate lost myocytes. In the study by Wang et al., a co-culture system was used by culturing β-actin GFP labeled ACM with neonatal cardiomyocytes. Not only 50% of the ACM remain alive as long as 7 days in culture with neonatal cardiomyocytes, by day 3, almost all the ACM lost organized sarcomere structure, suggesting these ACM start to enter their de-differentiation stage. Interestingly, coincident with the loss of the sarcomere structure, some of these ACM start to express cell proliferation marker genes, including Ki67 and PH3, providing further evidence that the adult mammalian cardiomyocytes can proliferate, at least in vitro following de-differentiation.
Lower vertebrates, such as zebrafish has been shown to preserve regenerative capacity in adult heart (8). Following apex resection, zebrafish cardiomyocytes will undergo a limited dedifferentiation process highlighted by disassembly of sarcomeric structure before re-entering cell cycle (9,10). This fascinating regenerative capacity also seems to be preserved in neonatal mammalian heart where cardiomyocytes from post-natal day 1 mouse heart can re-enter cell cycle and generate new heart muscle through proliferation following injury (11). Unfortunately, this regenerative capacity is largely lost on day 7. In this current study, Wang et al. used time-lapse video microscopy to record and clearly demonstrate that isolated adult mammalian cardiomyocytes also undergo a similar DPR process upon co-culturing with neonatal myocytes. Cardiomyocytes disassemble their sarcomere structure followed by cell cycle entry and proliferation to generate progenies, and finally re-differentiate into functional cardiomyocytes with organized sarcomere structure. Interestingly, this re-differentiation process appears to require direct contact between the co-cultured ACM and the neonatal cardiomyocytes, as well as propagation of Ca2+ transients from the neonatal cardiomyocytes. Manipulating the expression level of calcium handling apparatus in neonatal cardiomyocytes, including inhibiting SERCA2a and NCX1, can significantly impair sarcomere structure assembly in the newly generated ACM. These elegantly designed studies revealed for the first time the importance of cell-cell interaction and calcium propagation between cardiomyocytes in the regeneration of ACM. This discovery, together with another recent report that inactivation of Hippo pathway can promote post-mitotic cardiomyocyte proliferation and improve cardiac function following MI (12), really shed new light on novel paths to achieve cardiac regeneration in adult heart.
In addition to the proof-of-concept observation that in an in vitro co-culture system, the ACM hold the capacity of DPR, Wang et al. further provided evidence that, in intact mammalian heart, DPR also occurs in the infarct border zone 3 weeks post MI. Using a lineage tracing mouse line, Wang et al. have demonstrated the existing cardiomyocytes in the infarct border zone are positive for cell proliferation markers, even though the majority of these newly generated cells do not have detectable sarcomeric structure, suggesting these cells remain at undifferentiated stage and cannot contribute to the overall contractile function of the injured myocardium. This problem was attributed to impaired cell-cell junction in infarcted myocardium due to the loss of Cx43 expression and proper distribution, a well-established phenomenon in injured heart. However, Wang et al. provided a solution to this problem by expressing an ischemia-resistant Cx43 mutant through AAV9 mediated gene transfer. This phosphomimetic mutant of Cx43-Cx43-S3E is resistant to ischemia induced degradation and redistribution, and thus preserves gap junction following cardiac ischemia. Remarkably, by overexpressing this Cx43 ischemia resistant mutant, the mice showed significantly decreased infarction size 3 days post MI, together with improved cardiac function and less cardiac dilation 6 weeks post MI. This outcome supports the potential role of Cx43 mediated calcium propagation in promoting adult cardiomyocyte re-differentiation into functional myocardium (Figure 1).
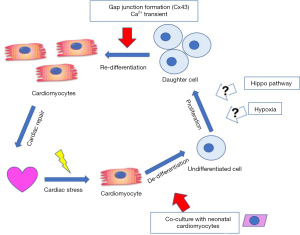
This interesting finding raises another question—what is the role of hypoxia during cardiac regeneration after injury? The current paradigm in the field is: oxygen metabolism and oxidative stress are critical regulators for the proliferative capacity of mammalian cardiomyocytes. Therefore, reducing oxygen metabolism through a hypoxia environment in adult mammalian heart can induce cardiomyocyte cell cycle re-entry through blunting oxidative stress and DNA damage (13,14). However, hypoxia clearly plays another role during the ACM re-differentiation into functional cardiomyocyte. According to Wang et al., hypoxia induces dephosphorylation of Cx43, impairing its function as a gap junction protein, therefore hampers the re-differentiation potential of ACM. Indeed, while expressing Cx43 phospho-dead mutant exacerbated hypoxia-induced loss of cardiomyocyte re-differentiation; expression of Cx43 phosphomimetic mutant enhanced the re-differentiation efficiency of ACM in vitro and potentially in vivo as well. One postulation is that—hypoxia plays different roles at different steps of the ACM DPR process. Initially, reducing oxygen metabolism can promote cell cycle re-entry of ACM by alleviating oxidative DNA damage, once cardiomyocytes enter the re-differentiation stage; on the other hand, hypoxia environment becomes detrimental due to impaired gap junction and electric coupling.
Another caveat of the current study is: although it is encouraging to see the functional recovery and the reduction of cardiac dilation post Cx43-S3E expression in vivo, it is unclear how much of the functional improvement indeed was contributed by the newly generated cardiomyocytes. This issue need to be further tested in a more rigorous way by combination of both in vivo and in vitro model.
Future development of therapy should consider the complexity of hypoxic signaling at different stages of myocardial repair in order to achieve most optimum therapeutic outcome. In the end, as the study by Wang et al. and others illustrate time and time again, cardiac regeneration is a complex process. There will be more findings needed with better molecular details and higher temporal resolution before the ultimate success of cardiac regeneration as a future therapy for heart failure.
Acknowledgements
The author would like to thank Dr. Yibin Wang (UCLA) for proof reading and suggestions.
Funding: This work is supported by American Heart Association Postdoctoral Fellowship (17POST33661136).
Footnote
Provenance and Peer Review: This article was commissioned and reviewed by the Section Editor Dr. Ziwei Li (Department of Surgery, The First Hospital Affiliated to Kunming Medical School, Kunming, China).
Conflicts of Interest: The author has completed the ICMJE uniform disclosure form (available at http://dx.doi.org/10.21037/amj.2018.01.05). The author has no conflicts of interest declare.
Ethical Statement: The author is accountable for all aspects of the work in ensuring that questions related to the accuracy or integrity of any part of the work are appropriately investigated and resolved.
Open Access Statement: This is an Open Access article distributed in accordance with the Creative Commons Attribution-NonCommercial-NoDerivs 4.0 International License (CC BY-NC-ND 4.0), which permits the non-commercial replication and distribution of the article with the strict proviso that no changes or edits are made and the original work is properly cited (including links to both the formal publication through the relevant DOI and the license). See: https://creativecommons.org/licenses/by-nc-nd/4.0/.
References
- He L, Zhou B. Cardiomyocyte proliferation: remove brakes and push accelerators. Cell Res 2017;27:959-60. [Crossref] [PubMed]
- Morikawa Y, Heallen T, Leach J, et al. Dystrophin-glycoprotein complex sequesters Yap to inhibit cardiomyocyte proliferation. Nature 2017;547:227-31. [Crossref] [PubMed]
- Magadum A, Ding Y, He L, et al. Live cell screening platform identifies PPARδ as a regulator of cardiomyocyte proliferation and cardiac repair. Cell Res 2017;27:1002-19. [Crossref] [PubMed]
- Wang WE, Li L, Xia X, et al. Dedifferentiation, Proliferation, and Redifferentiation of Adult Mammalian Cardiomyocytes After Ischemic Injury. Circulation 2017;136:834-48. [Crossref] [PubMed]
- Canseco DC, Kimura W, Garg S, et al. Human ventricular unloading induces cardiomyocyte proliferation. J Am Coll Cardiol 2015;65:892-900. [Crossref] [PubMed]
- Bergmann O, Bhardwaj RD, Bernard S, et al. Evidence for Cardiomyocyte Renewal in Humans. Science 2009;324:98-102. [Crossref] [PubMed]
- Mollova M, Bersell K, Walsh S, et al. Cardiomyocyte proliferation contributes to heart growth in young humans. Proc Natl Acad Sci U S A 2013;110:1446-51. [Crossref] [PubMed]
- Poss KD, Wilson LG, Keating MT. Heart Regeneration in Zebrafish. Science 2002;298:2188-90. [Crossref] [PubMed]
- Jopling C, Sleep E, Raya M, et al. Zebrafish heart regeneration occurs by cardiomyocyte dedifferentiation and proliferation. Nature 2010;464:606-9. [Crossref] [PubMed]
- Kikuchi K, Holdway JE, Werdich AA, et al. Primary contribution to zebrafish heart regeneration by gata4+ cardiomyocytes. Nature 2010;464:601-5. [Crossref] [PubMed]
- Porrello ER, Mahmoud AI, Simpson E, et al. Transient Regenerative Potential of the Neonatal Mouse Heart. Science 2011;331:1078-80. [Crossref] [PubMed]
- Leach JP, Heallen T, Zhang M, et al. Hippo pathway deficiency reverses systolic heart failure after infarction. Nature 2017;550:260-4. [PubMed]
- Kimura W, Nakada Y, Sadek HA. Hypoxia Induced Myocardial Regeneration. J Appl Physiol 2017; [Epub ahead of print]. [Crossref] [PubMed]
- Nakada Y, Canseco DC, Thet S, et al. Hypoxia induces heart regeneration in adult mice. Nature 2017;541:222-7. [Crossref] [PubMed]
Cite this article as: Gao C. Adult cardiomyocyte regeneration: if I could ever travel back time. AME Med J 2018;3:16.